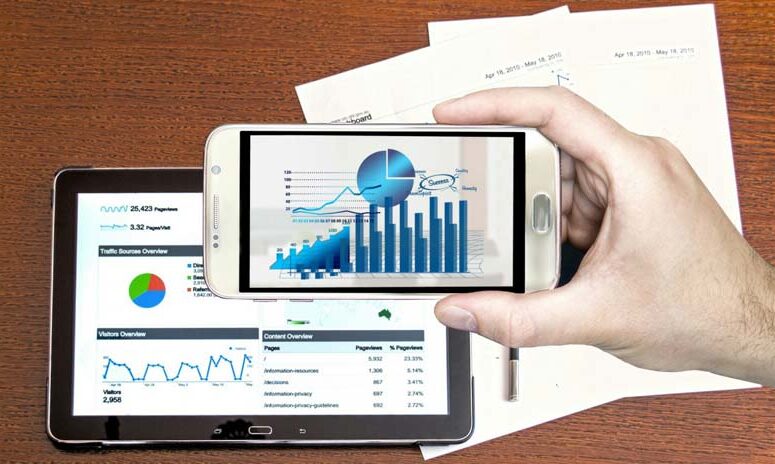
Image Credit: pxhere.com
3D bioprinting is advancing rapidly, drawing the attention of researchers, clinicians, and biotech professionals eager to harness its potential to fabricate living tissues and organs. Laboratories worldwide are exploring how to accurately print viable cells in complex architectures, aiming for breakthroughs in regenerative medicine and drug development. As the field moves toward higher fidelity and more sophisticated materials, the demand for meticulous data gathering and analysis grows ever more pressing. JavaScript Charts are frequently employed by many development teams on the first page of their data-analysis toolkit to interpret and visualize the myriad metrics tied to 3D bioprinting projects.
One developer from SciChart advises that improving visual feedback loops can significantly cut trial-and-error time and speed up innovation. He remarks that in fast-paced sectors such as this, teams benefit from advanced rendering solutions, especially JavaScript 3D Charts, which offer precision and scalability when dealing with large datasets.
This article focuses on the key success metrics of 3D bioprinting and how up-to-date visualization technologies can map these factors in a comprehensive and accessible way. Emerging approaches in 3D bioprinting are yielding better outcomes, but only when data is measured, understood, and used to make informed decisions. Getting to grips with the relevant metrics, however, remains a challenge. From cell viability and structural fidelity to the mechanical properties of printed constructs, each parameter carries significance for the ultimate efficacy of bioprinted tissues.
Table of Contents
3D Bioprinting in Context
3D bioprinting does not just stack layers of inert material; it integrates living cells within a scaffold or bio-ink to produce functional tissue-like constructs. Researchers strive to replicate the complex microenvironment of body tissues, prompting innovations in scaffold design, printing resolution, and the fine-tuning of hydrogels. The process typically involves selecting a suitable bio-ink composition, optimizing print settings for cell health, and cultivating the printed construct under conditions that encourage tissue formation.
Success in this arena can be measured by looking at multiple dimensions. There is the immediate concern of how cells behave during and after the printing process, followed by the long-term maturation of the printed construct. These factors can be influenced by printing pressure, nozzle diameter, extrusion speed, and material composition. Each printing approach carries unique trade-offs, with scientists debating the merits of extrusion-based, inkjet-based, or laser-assisted methods. The ideal printing mechanism depends on the target tissue type, the fragility of the cells involved, and the complexity of the final structure.
High-Resolution Data Acquisition
Before examining metrics such as fidelity or viability, practitioners must capture data at every step. This starts with controlling parameters like temperature, shear force, and printing speed to preserve cell integrity. Instruments that measure real-time conditions help confirm whether each droplet or filament of bio-ink is consistent with the intended design. Post-print, imaging technologies such as confocal microscopy or live-cell imaging reveal the distribution of cells and their level of survival within the construct.
The depth of data is astounding. Early designs of 3D bioprinting might have considered only a handful of factors, but modern approaches can accumulate dozens of variables for each small section of printed tissue. This wealth of information can guide iterative refinement, but only if it is organized and analyzed effectively. Traditional spreadsheets and static graphs can no longer match the complexity of this domain, leading to growing adoption of dynamic, interactive visualization systems. These systems make it possible to overlay multiple layers of information—such as temperature gradients, mechanical properties, and growth patterns—on top of each other in three-dimensional space.
The Importance of Structural Fidelity
One of the first visible indicators of success is how faithfully the printed construct matches its intended design. Structural fidelity captures metrics like layer thickness, pore size in scaffolds, and dimensional accuracy. Discrepancies occur when bio-inks behave unpredictably under nozzle pressure or if cells do not remain where they are placed due to the fluidic nature of certain materials. If a scaffold collapses or warps, the tissue may fail to develop correct vascularization or mechanical strength.
Researchers often define a shape or geometry that the printed tissue must achieve. Advanced visualization tools permit cross-sectional and volumetric comparisons of the final print versus the digital blueprint. High-resolution data from imaging devices can be fed into specialized software to generate color-coded deviation maps indicating regions that diverge from the intended design. Even slight deviations in pore size can matter, as they influence nutrient exchange and the mechanical characteristics of the final construct.
Cell Viability and Proliferation
Whether working with stem cells, cardiomyocytes, or other specialized cells, viability measures are critical. The printing process can impose stress on cells, reducing their survival rate. Monitoring immediate viability can highlight whether the chosen method is too harsh. Longer-term proliferation rates then indicate if the cells can multiply and form functional networks.
Data surrounding cell viability ranges from basic live-dead assays to more complex analyses of specific markers of functionality. Automated image analysis can detect fluorescent signals marking living or apoptotic cells, producing large datasets requiring sophisticated visual interpretation. Tracking the proliferation trend over time reveals whether the cells adapt well to their new environment. If cells exhibit low viability after printing, optimization must follow—altering nozzle configurations, adjusting pressure or temperature, or changing bio-ink ingredients.
Biological Functionality
Printing living cells in three dimensions is only the start. For many applications—especially regenerative medicine—bioprinted tissues must perform the biological functions of real tissue. Researchers observe markers indicating whether cells differentiate or secrete key proteins. They might measure contractile force in cardiac patches or test the synaptic connectivity of neural constructs. These specific indicators attest to the tissue’s capability to integrate into the body or serve as an accurate in vitro model.
Monitoring and quantifying such biological functionality is more challenging than measuring dimensions or counting cell numbers. Techniques range from electrical impedance measurements to gene expression profiling, each producing a distinct data stream. A comprehensive approach links morphological data (structural fidelity and viability) with functional data (electrophysiological signals, molecular markers) to build a holistic picture. When these datasets are combined in three-dimensional visualizations, researchers can see how different regions of a printed tissue exhibit varying levels of functionality, pointing out hotspots for improvement.
Mechanical Integrity
Tissues in the human body experience continuous mechanical stress. Bone, cartilage, and even heart tissue must maintain structural integrity while supporting loads and dynamic forces. In 3D bioprinting, mechanical integrity hinges on the choice of materials and the printing pattern. Commonly, hydrogels are used for soft tissues, while synthetic polymers or composite materials are considered for bone scaffolds.
Testing mechanical properties reveals how well the printed construct can endure compression, bending, or stretching. Investigators measure compression moduli, tensile strength, and elasticity to confirm alignment with the intended biological tissue. If the construct falls short, the question becomes whether to modify the material or change the printing pattern. A strong yet porous structure can be achieved by designing lattice-like geometries that replicate the body’s natural architecture. Visual analytics help highlight load-bearing regions and any flaws that might cause premature failure.
Nutrient Diffusion and Vascularization
Vascularization is essential to sustain living cells in larger 3D constructs. Without sufficient nutrient exchange, tissues become necrotic at their core. Researchers often incorporate channels or vascular-like networks, sometimes seeding endothelial cells to encourage vessel formation. The success of these approaches is tied to diffusion rates of oxygen and nutrients, which can be modeled and validated experimentally.
Tracking nutrient gradients over time can clarify whether the printed design supports consistent nutrient delivery. Some teams rely on oxygen-sensing dyes or advanced imaging to watch how far oxygen penetrates into the tissue. Others measure consumption rates of glucose or amino acids. The data can be fed into computational fluid dynamics models, which are visualized in three dimensions to display potential areas of insufficient perfusion. Pinpointing nutrient bottlenecks allows for targeted interventions, such as modifying the channel network or adding growth factors to promote vascular sprouting.
Quality Control and Batch Reproducibility
Scaling up production of bioprinted tissues for clinical or pharmaceutical applications calls for rigorous quality control. A single misalignment or contaminant can undermine months of work, making reproducibility a top priority. Quality control encompasses verifying the structural fidelity, cell viability, and mechanical properties of each batch. Advanced data visualization can highlight statistical patterns across multiple prints, revealing subtle drifts in printing parameters or variations in material consistency.
Achieving reproducibility also depends on standardizing protocols. Automated systems that control temperature, humidity, and printing speeds can reduce human error. Data logs containing thousands of variables might be interpreted more efficiently using advanced charting solutions that offer real-time analysis. Researchers can track slight deviations in printing performance over weeks or months, intervening early if a drift suggests future defects. Consistency builds trust in the final product, especially if the printed tissues are intended for transplantation or high-stakes drug testing.
Regulatory Considerations
As 3D bioprinting moves toward clinical implementation, regulators demand detailed proof of safety, efficacy, and manufacturing consistency. Complex data repositories demonstrating control over the entire process must be maintained. In many jurisdictions, this includes demonstrating compliance with Good Manufacturing Practice (GMP), traceability of materials, and standardized testing methods.
Regulatory bodies tend to question how developers ensure printed tissues replicate a predictable micro-architecture and maintain cell viability. Visual charts can illustrate time-series data for each print, along with measurements of mechanical properties and growth rates. This not only speeds up the review process but also fosters transparency. If an adverse outcome occurs, the data can be traced back to identify the root cause. Hence, robust data visualization is not just a research tool; it becomes a cornerstone of regulatory documentation.
Adoption in Drug Screening
Beyond transplantation and regenerative aims, 3D bioprinting is popular in drug discovery. Bioprinted tissues can resemble native human tissues more closely than conventional 2D cell cultures, making them valuable for evaluating new compounds. If a prospective therapy shows promise in a bioprinted cardiac patch, the results are more likely to align with how the compound might behave in a real heart.
For drug developers, visualizing dose-response curves and toxicity data in three-dimensional space yields insights into how compounds disperse and affect different cell layers. Integrating real-time readouts of metabolic activity can create dynamic models that shift as the cells respond to the drug. The challenge lies in collecting and interpreting these large volumes of data, which may include multiple cell types co-cultured in a single construct.
Data Integration and Cloud-Based Collaboration
Large-scale projects in 3D bioprinting may be distributed across multiple departments or institutions. Sharing data in real time ensures that all stakeholders can monitor progress, compare notes, and propose adjustments. Cloud-based platforms have emerged where data is uploaded and visualized instantly, enabling remote teams to explore interactive models without local hardware limitations.
Collaboration at this level is a departure from the older method of emailing static images or spreadsheets. Instead, each collaborator can manipulate the same 3D charts, apply filters, and investigate anomalies. This fosters rapid feedback cycles and shortens the time from concept to a refined, functional tissue. When dealing with iterative work, teams rely on version control so they can revert to earlier configurations if necessary. The synergy between data collection, analysis, and discussion boosts the overall efficiency of bioprinting research.
Environmental and Sustainability Factors
Although overshadowed by medical potential, environmental considerations matter in 3D bioprinting. Some labs aim to reduce waste, energy consumption, or reliance on animal products in bio-inks. Metrics that track resource usage, print success rates, and disposal procedures highlight how “green” a bioprinting operation is. Visual analytics can show the life cycle of materials, from raw ingredients to final disposal, reinforcing a responsible approach.
Tracking sustainability metrics can also encourage innovative methods, such as using algal-based bio-inks or reclaimed biomaterials. If energy consumption spikes for a specific printing approach, data can shed light on whether a tweak in temperature or pressure might yield a more energy-efficient process. By correlating success rates with environmental impact, teams can optimize for both performance and sustainability. An environmentally conscious approach can further bolster the credibility of 3D bioprinting as a transformative medical technology.
Clinical Translation
The ultimate aim of many bioprinting initiatives is to produce tissues that integrate seamlessly into the human body. Clinical translation involves bridging the gap between controlled lab environments and the unpredictable realities of patient care. In clinical settings, success metrics become more patient-centric, focusing on how the printed tissue performs post-implantation. Does it vascularize quickly? Is the immune response minimized? Does the tissue carry out the intended function (e.g., beating in synchrony for cardiac patches)?
Each phase of clinical testing involves robust data analysis, from pre-clinical animal studies to initial human trials. These complex data sets cover everything from immunological markers to quality-of-life improvements for patients. Standard operating procedures incorporate real-time monitoring of the graft or implant, often employing imaging technologies such as MRI or CT scans to observe the integration of the printed tissue. The data is used to refine the design for future cohorts or re-engineer the bio-ink composition if complications arise.
Harnessing the Power of Dynamic Charts
Cutting-edge charting libraries allow researchers to delve into complex data sets with minimal friction. Having the ability to rotate 3D visualizations, zoom in on particular data points, and toggle specific parameters fosters a deeper understanding of the trends. Because real-world data is seldom neat or complete, interactive filters help isolate outliers or fill gaps in a reasoned manner.
Beyond presenting data, dynamic charts can incorporate computational models that predict how a tissue might develop. Investigators can simulate how changes to nutrient channels or mechanical constraints alter the final outcome, seeing results in a matter of seconds rather than weeks. Such synergy of modeling and real data paves the way for predictive analytics, in which data streams inform real-time adjustments to printing parameters. This closed-loop approach signals a future where the printer can adapt on the fly to ensure optimum tissue quality.
Optimizing Parameters with Machine Learning
Machine learning (ML) has found its way into 3D bioprinting research as teams look for ways to optimize printing protocols. Traditional approaches rely on trial and error, but ML algorithms can process large data sets of printing conditions and outcomes, identifying relationships that humans might overlook. When integrated with advanced charting solutions, these models can be visualized in a user-friendly manner.
For example, a research team might feed an algorithm data from hundreds of prints, labeling each print’s success based on cell viability and structural fidelity. The ML system then suggests printing parameters most likely to yield higher success. Monitoring its suggestions in real time on an interactive chart can show how changes in nozzle diameter might interact with different cell densities. Over multiple iterations, the system refines its predictions, offering an increasingly precise set of instructions. This type of data-driven feedback loop lowers material costs and expedites discovery.
Economic Considerations in 3D Bioprinting
Though 3D bioprinting commands scientific fascination, it must also be economically viable. Hospitals and biotech companies view bioprinting as an investment, gauging not just purchase and operating expenses but also throughput, potential revenue streams, and time to market. Success metrics in this context extend beyond biology, encompassing productivity rates and cost analysis of each printed unit.
Charting these economic factors alongside biological metrics can reveal trade-offs. A slightly more expensive bio-ink might yield better cell survival, reducing wasted prints and expensive lab time. Conversely, an economically efficient approach that compromises on mechanical stability may lead to costly failures down the line. Visual analytics integrating finance and research data help decision-makers identify the best balance. In practice, this might mean adopting a high-end printer for complex constructs and a simpler, cheaper alternative for routine tasks.
Open Innovation and Collaborative Platforms
The complexities of 3D bioprinting often exceed the capabilities of a single institution. Collaborative platforms, supported by advanced visualization, encourage sharing of printing protocols, material formulations, and data sets. By pooling knowledge, researchers can accelerate progress and reduce duplication of effort. Public repositories sometimes allow participants to compare results side by side, identifying best practices or recurring flaws.
Such open innovation frameworks benefit from uniform data standards. If each lab uploads information in a common format, global comparisons become feasible. This can drive consensus on what benchmarks define success for different tissue types, clarifying the metrics to track and how to measure them. More standardized data sets also attract interest from software developers, who can refine visual analytics tools to cater specifically to 3D bioprinting needs. Over time, the synergy of open science and specialized software fosters a vibrant ecosystem that evolves in step with new discoveries.
Further Expanding Capabilities with Multi-Material Printing
An emerging trend in bioprinting is the use of multiple materials in a single print, which more closely emulates the complex structures found in the human body. A construct might combine a stiff material for load-bearing sections and a softer gel where high cell viability is paramount. Manipulating these various compositions adds another layer of data complexity. Each material reacts differently to printing parameters such as extrusion speed and temperature.
Multi-material printing heightens the importance of advanced visualization because each region of the construct can have different mechanical or biological properties. Charts that delineate these properties by material type help ensure consistency throughout the print. Failure to manage this complexity could result in areas of the construct that are too rigid, limiting nutrient diffusion or causing localized stress. Visual analytics identify these mismatches swiftly, guiding refinements for improved integration of diverse materials.
Ethical Considerations
While 3D bioprinting offers enormous potential, ethical questions arise around creating living tissues. Some worry about inadvertently pushing the boundaries of what is considered acceptable in regenerative medicine, especially if printed organs become commercially driven products. In parallel, the confidentiality of patient data and biomaterials must be protected if they are used in personalized printing.
Part of ethical stewardship involves maintaining transparency about the reliability and safety of bioprinted constructs. The data used to validate these constructs must be both accurate and accessible to relevant stakeholders, including patients, regulatory agencies, and medical professionals. Advanced charts can serve this transparency goal by illustrating how each tissue is made, tested, and validated, ensuring decisions are made on a foundation of verifiable evidence.
Challenges and Opportunities Ahead
Developers continue to refine printing methods, exploring new materials like decellularized extracellular matrices or synthetic polymers that better mimic the body’s natural environment. Challenges persist around scaling up production, ensuring reproducible vascularization, and integrating neural networks. Another notable challenge is bridging the gap between lab-based successes and commercial viability, a journey that can be shortened by robust data management systems.
Data visualization that can toggle between micro-level and macro-level perspectives will be a game-changer. At the micro level, scientists focus on a single layer or cluster of cells. At the macro level, hospital administrators and biotech companies assess the cost-effectiveness and therapeutic impact across a patient population. Navigating these layers demands multi-faceted charts that cater to both scientific depth and managerial oversight. Innovative charting libraries push these boundaries by supporting real-time data inputs, customizable analytics, and aesthetically clear designs.
Continual Innovation in Data Visualization
As hardware and software tools evolve, charting libraries must adapt. New data types, such as volumetric imaging or real-time sensor readings, call for flexible visualization components. The next generation of JavaScript Charts, for example, may incorporate virtual reality (VR) or augmented reality (AR) to let users immerse themselves in the data, rotating and probing 3D constructs in virtual space.
Such immersive experiences could streamline collaboration by allowing researchers in different locations to interact with the same 3D model simultaneously. Voice commands or gesture controls could annotate specific sections of the construct, suggesting modifications for the printing process. While this might sound futuristic, early prototypes hint that VR-based analytics can indeed spark more intuitive data exploration. As with all innovations, ensuring stable performance, minimal latency, and cross-platform compatibility remains paramount.
Paving the Road to Mainstream Adoption
For 3D bioprinting to become a mainstream medical solution, broader acceptance and cost-effectiveness must align with regulatory assurances of safety and efficacy. Interactive charts play an essential role in communicating these assurances, demonstrating a chain of data from initial design to final outcome. This builds confidence among investors, researchers, and clinicians alike, reducing perceived risks in embracing new technologies.
Educational institutions are also increasingly integrating 3D bioprinting and related data analytics into their curricula, fostering a new generation of professionals adept at interpreting complex data sets. As these graduates enter the workforce, their familiarity with advanced charting techniques helps push the field forward. Ultimately, the synergy between technical expertise, reliable data visualization, and robust printing methods sets the stage for 3D bioprinting’s sustainable growth.
Conclusion
3D bioprinting stands at the convergence of biology, engineering, and data science. Its success is defined by a range of metrics, from the structural and mechanical fidelity of printed constructs to the viability, functionality, and reproducibility of embedded cells. Regulatory concerns, ethical questions, and economic factors add further layers of complexity. Central to all these considerations is the effective collection, organization, and visualization of data.
When researchers and developers employ advanced charting solutions, they can better map each step of the 3D bioprinting journey, pinpointing areas for improvement and validating breakthroughs. The real promise lies in bridging these multiple domains—biology, chemistry, physics, engineering, and even machine learning—to craft living tissues that not only survive but thrive in complex biological environments. As the technology continues to evolve, so too will the methods for scrutinizing the ocean of data it generates, driving 3D bioprinting toward its goal of transforming healthcare and research on a global scale.
Also Read: Cross-Platform Development with Kotlin Multiplatform: Bridging the Gap Between Android and iOS